당신은 주제를 찾고 있습니까 “the ventilator book pdf – The best ICU book references for new providers“? 다음 카테고리의 웹사이트 you.tfvp.org 에서 귀하의 모든 질문에 답변해 드립니다: you.tfvp.org/blog. 바로 아래에서 답을 찾을 수 있습니다. 작성자 Bree Juskowiak 이(가) 작성한 기사에는 조회수 1,374회 및 좋아요 44개 개의 좋아요가 있습니다.
Table of Contents
the ventilator book pdf 주제에 대한 동영상 보기
여기에서 이 주제에 대한 비디오를 시청하십시오. 주의 깊게 살펴보고 읽고 있는 내용에 대한 피드백을 제공하세요!
d여기에서 The best ICU book references for new providers – the ventilator book pdf 주제에 대한 세부정보를 참조하세요
The 5 must have and 2 nice to have books for a successful rotation or onboarding in ICU as a provider. These are the tried and true books that most everyone I know has used. I only suggest books that are easy to read, understand, and interpret.
If you need to purchase any of these and you want to do so on Amazon I would love if you’d use my affiliate links below. TYSM!
The ICU Book by Paul Marino https://amzn.to/3jbh2vn
(A must have. Easy to read, literally cover to cover. Also a life-long reference. Particularly helpful when the nurse calls you for tachycardia – simply flip to that page, it gives you actionable sequence of events with a little bit of pathophys)
The Ventilator Book https://amzn.to/2UvENE0
(A must have)
The Advanced Ventilator Book: https://amzn.to/3nqL4Nq
Essentials of Mechanical Ventilation https://amzn.to/3jiOXCi
(Deeper understanding, does a good job describing the mechanics and also I love how they break down waveforms)
The Ultimate H\u0026P Cheatsheet: https://www.breenp.com/shop
Rapid Interpretation of EKG: https://amzn.to/3oCC1Zb
Chest X-Ray Interpretations: https://amzn.to/3wXpZ06
If you’d like to visit my website to see what consulting services I offer here is the link: https://www.breenp.com/
The opinions shared here are my own and may not reflect those of my employers. This is educational and does not constitute medical advise.
the ventilator book pdf 주제에 대한 자세한 내용은 여기를 참조하세요.
The Ventilator Book 3ed 2021 William Owens | Medical Books
The Ventilator Book has been the go-to reference for physicians, … Title: The Ventilator Book; Author: William Owens … Ebook PDF; File size: 8.3 MB.
Source: medbookvn.com
Date Published: 3/16/2022
View: 6941
The Ventilator Book – Critical Care
The second part of the book is intended to teach you about mechanical ventilation. The chapters are short, and each can be read easily within 15-20 minutes.
Source: criticalcare.cooperhealth.org
Date Published: 4/24/2022
View: 8818
The Ventilator Book
Commandments of Mechanical Ventilation. The second part of the book is intended to teach you about mechanical ventilation. The chapters are short, and each …
Source: n2ncollection.com
Date Published: 7/18/2022
View: 6824
The Ventilator Book by William Owens – PDF Drive
The Advanced Ventilator Book is a companion to the best-selling The Ventilator Book and is written for clinicians who al .
Source: www.pdfdrive.com
Date Published: 3/9/2021
View: 495
The Ventilator Book [3rd Edition] 0985296569, 9780985296568
The third edition of The Ventilator Book combines the content of the original book with key chapters from The Advanced V… … Commentary; CONVERTED PDF.
Source: dokumen.pub
Date Published: 9/13/2022
View: 4868
Mechanical Ventilation
This book is dedicated to the resents and fellows that have struggled in the ICU and to the patients who have struggled with them. Page 5. Notice. Legalese I …
Source: www.orlandocriticalcare.com
Date Published: 6/12/2022
View: 248
주제와 관련된 이미지 the ventilator book pdf
주제와 관련된 더 많은 사진을 참조하십시오 The best ICU book references for new providers. 댓글에서 더 많은 관련 이미지를 보거나 필요한 경우 더 많은 관련 기사를 볼 수 있습니다.
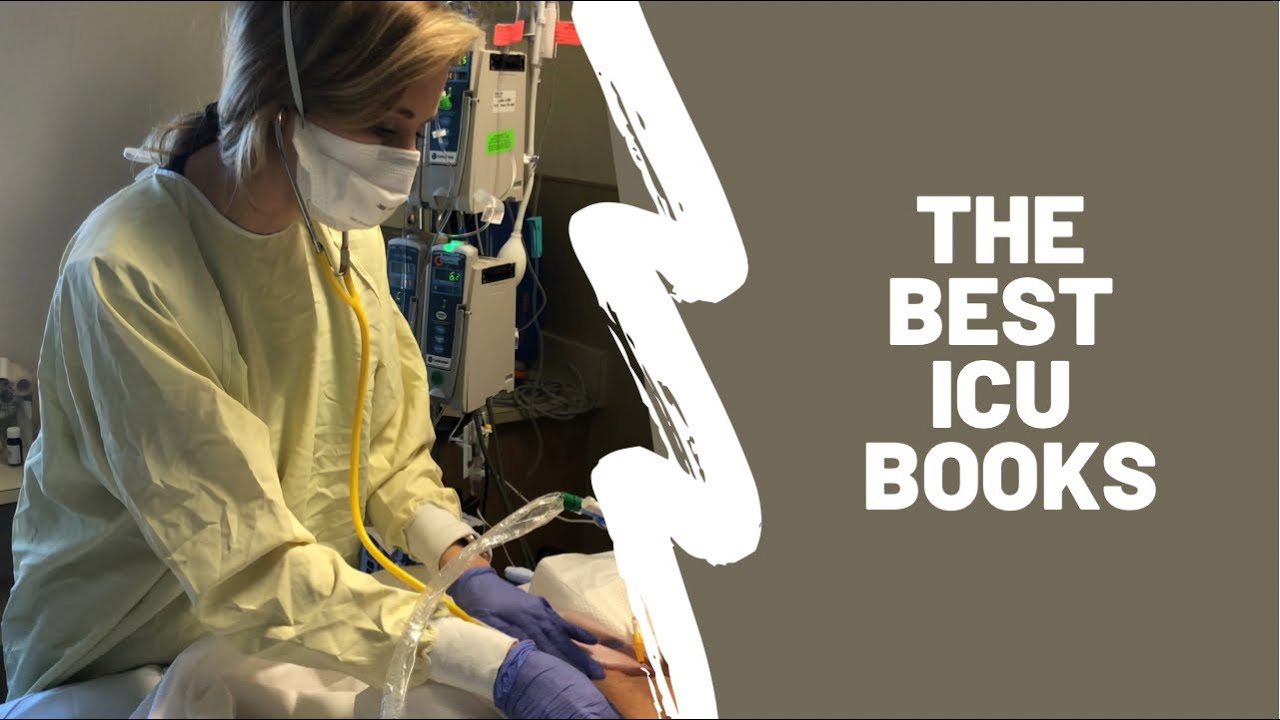
주제에 대한 기사 평가 the ventilator book pdf
- Author: Bree Juskowiak
- Views: 조회수 1,374회
- Likes: 좋아요 44개
- Date Published: 2021. 11. 19.
- Video Url link: https://www.youtube.com/watch?v=uHpnz56qr1I
The Ventilator Book 3ed 2021 William Owens
DESCRIPTION
The third edition of The Ventilator Book combines the content of the original book with key chapters from The Advanced Ventilator Book into one comprehensive reference.
The Ventilator Book has been the go-to reference for physicians, advanced practice providers, respiratory therapists, fellows, residents, and students working in the Intensive Care Unit since 2012. It has been published in four languages, with over 50,000 copies in print.
Dr. William Owens explains, in clear language, the basics of respiratory failure and mechanical ventilation. This is a guide to keep in your jacket pocket, call room, or in the ICU.
Chapters have been updated to reflect new developments in critical care medicine and the experience gained during the COVID-19 pandemic. The book is divided into sections on physiology and technology; conventional modes and basic concepts; and unconventional modes and advanced concepts. As always, there are chapters for initial ventilator setup, adjustments, and troubleshooting. Patient-ventilator dyssynchrony, rescue therapies for ARDS, and ECMO are also covered.
The goal of The Ventilator Book is to demystify mechanical ventilation for the nonexpert practitioner and to emphasize safe, patient-based critical care. This edition lives up to the intent of the best-selling original, which is to make difficult concepts easy to understand.
DETAILS
Title: The Ventilator Book
Author: William Owens
Publisher: First Draught Press
Publication: 2021
Edition: 3
Language: English
Pages: 301 Ebook PDF
File size: 8.3 MB
REVIEWS
I am an ICU RN. Probably many of those things explained in this book I was supposed to know, but I didn’t. Talks between an RT and a physician were a mystery to me many times. Now I understand their reasoning behind decisions. I gained lots of confidence by reading the book that was very easy to read. It is also written quite artistically, and with a lot of sense of humor! Absolutely priceless book!
— Larisa Rudelson
Xem thêm:
The Ventilator Book by William Owens
The Advanced Ventilator Book
75 Pages · 2017 · 2.14 MB · 2,339 Downloads · New!
The Advanced Ventilator Book is a companion to the best-selling The Ventilator Book and is written for clinicians who al …
The Ventilator Book [3rd Edition] 0985296569, 9780985296568
Table of contents :
Title Page……Page 3
Copyright Page……Page 4
Dedication……Page 5
Table of Contents……Page 6
Introduction……Page 8
Philosophy of Mechanical Ventilation……Page 10
Chapter 1: Initial Settings……Page 13
Chapter 2: Quick Adjustments and Troubleshooting……Page 21
Chapter 3: The Eleven Commandments of Mechanical Ventilation……Page 29
Chapter 4: Acute Respiratory Failure……Page 35
Chapter 5: Oxygen Delivery and Consumption……Page 46
Chapter 6: Permissive Hypercapnia……Page 58
Chapter 7: Monitoring of the Ventilated Patient……Page 63
Chapter 8: Safety Limits and Lung Protection……Page 73
Chapter 9: Assist-Control Ventilation……Page 90
Chapter 10: Synchronized Intermittent Mandatory Ventilation……Page 100
Chapter 11: Pressure Support Ventilation……Page 104
Chapter 12: CPAP, PEEP, and Optimal PEEP……Page 109
Chapter 13: Trigger and Flow……Page 127
Chapter 14: Patient-Ventilator Dyssynchrony……Page 135
Chapter 15: Severe Bronchospasm and Hyperinflation……Page 149
Chapter 16: Prone Positioning and Neuromuscular Blockade……Page 163
Chapter 17: Airway Pressure Release Ventilation……Page 172
Chapter 18: Inhaled Pulmonary Vasodilators……Page 180
Chapter 19: High Frequency Oscillatory Ventilation……Page 190
Chapter 20: Veno-Venous ECMO……Page 196
Chapter 21: Veno-Arterial ECMO……Page 205
Chapter 22: Liberation from Mechanical Ventilation……Page 212
Chapter 23: Prolonged Respiratory Failure……Page 217
Chapter 24: Mechanical Ventilation During a Pandemic or Mass Casualty Event……Page 226
Chapter 25: Seven Rules for Severe Respiratory Failure……Page 240
Chapter 26: Ventilator Flowsheets and Guidelines……Page 247
Appendix of Useful Formulae……Page 265
References……Page 268
Acknowledgements……Page 278
About the Author……Page 279
Citation preview
Medicine is an ever-changing discipline, and the subject matter of this book is no exception. While the author has done his best to ensure that this book reflects contemporary evidence-based practice, new developments in the field may supersede the material published here. Only properly trained and licensed practitioners should provide medical care to patients with respiratory failure. Nothing in this book should be construed as advice regarding the care of a specific patient or group. Copyright © 2021, 2018, 2012 by William Owens, MD All rights reserved. This book or any portion thereof may not be reproduced or used in any manner whatsoever without the express written permission of the publisher except for the use of brief quotations in a book review. Third Edition Cover Design by Lorien Owens Published by First Draught Press Columbia, SC ISBN 978-0-9852965-6-8 Printed in the United States of America
To Lorien—my wife, best friend and fellow adventurer, who has stood by me through thick and thin for nearly 20 years. You are the one who encouraged me to write the first edition in Pittsburgh, and you have given me the necessary prodding to write the second and third editions as well. I couldn’t have done this without you. William, Zach, and Amelia—you three are the best kids I could ever hope to have. Not many families have conversations like ours, and I’m pleased that all three of you know what a ventilator is and the indications for ECMO. I’m proud to be the dad of three happy, independent, naturally curious people who are all determined to make their marks on the world.
Table of Contents Introduction Philosophy of Mechanical Ventilation
QUICK REFERENCE GUIDE Chapter 1: Initial Settings Chapter 2: Quick Adjustments and Troubleshooting
PHYSIOLOGY AND TECHNOLOGY Chapter 3: The Eleven Commandments of Mechanical Ventilation Chapter 4: Acute Respiratory Failure Chapter 5: Oxygen Delivery and Consumption Chapter 6: Permissive Hypercapnia Chapter 7: Monitoring of the Ventilated Patient Chapter 8: Safety Limits and Lung Protection
CONVENTIONAL MODES AND BASIC CONCEPTS Chapter 9: Assist-Control Ventilation Chapter 10: Synchronized Intermittent Mandatory Ventilation Chapter 11: Pressure Support Ventilation Chapter 12: CPAP, PEEP, and Optimal PEEP Chapter 13: Trigger and Flow Chapter 14: Patient-Ventilator Dyssynchrony Chapter 15: Severe Bronchospasm and Hyperinflation
UNCONVENTIONAL MODES AND ADVANCED CONCEPTS
Chapter 16: Prone Positioning and Neuromuscular Blockade Chapter 17: Airway Pressure Release Ventilation Chapter 18: Inhaled Pulmonary Vasodilators Chapter 19: High Frequency Oscillatory Ventilation Chapter 20: Veno-Venous ECMO Chapter 21: Veno-Arterial ECMO
NEXT STEPS Chapter 22: Liberation from Mechanical Ventilation Chapter 23: Prolonged Respiratory Failure
USEFUL STUFF Chapter 24: Mechanical Ventilation During a Pandemic or Mass Casualty Event Chapter 25: Seven Rules for Severe Respiratory Failure Chapter 26: Ventilator Flowsheets and Guidelines Appendix of Useful Formulae References Acknowledgements About the Author
Introduction So, here you are in the Intensive Care Unit at 3:30 in the morning. The Emergency Department has just admitted a patient to your service—a young man with a rather sudden onset of fever, rigors, and respiratory distress. He had to be intubated in the ED and the ventilator seems to be alarming with a nerve-racking frequency. His chest X-ray looks horrible, with diffuse infiltrates and consolidations. The ICU respiratory therapist looks at you and asks the question you have been dreading since the patient arrived— “Doctor, what vent settings do you want?” This is a familiar story for those of us who spend a lot of time in the ICU, and since the advent of the COVID-19 pandemic, it’s an experience that just about every student and resident has at least once during his or her training. Mechanical ventilation can be intimidating—it has its own terminology, not all of which makes sense; it’s a life-sustaining technology, and misapplication can have serious consequences; and practitioners of mechanical ventilation tend to talk in esoteric ways about what the ventilator is doing. This can confuse even the smartest practitioner. To make things worse, there aren’t a lot of practical resources for busy clinicians who just need some quick guidance on how to adjust the ventilator. Don’t get me wrong—there are plenty of great textbooks on mechanical ventilation. And, if you have the time, they are well worth reading. The operative word, however, is “time.” Reading a hundred pages on the pros and cons of pressure-controlled ventilation may be a good use of an afternoon in the library but it’s wholly impractical while taking care of patients in a busy ICU. What’s necessary is a how-to guide, and that’s why I first wrote this book in 2012. It may seem basic to experienced ICU clinicians, but then again, it’s written for the nonexperts in the room. I would also venture to say that most success in critical care medicine comes from doing the basic stuff right on a consistent basis. This is now the third edition of The Ventilator Book, which I have enjoyed putting together. I have combined some new material with updated chapters from the second edition of The Advanced Ventilator Book to create a
more comprehensive single resource. I would like to thank many of my readers for their feedback on previous editions, and I hope you’ll find that it had an impact on this one. Since there’s only one author, this book will be biased. Not too much, I hope, but I’m not delusional enough to think that my approach is completely objective. Like everyone else in medicine, personal anecdote and experience has shaped my practice. This book is intended to teach you about the practical aspects of mechanical ventilation. The chapters are short, and each can be read easily within 15-20 minutes. Here, you’ll learn to speak the language and understand the rationale for why things work and why intensivists do what they do. I also believe that understanding the “whys” of what we do is important, and I’ve included some chapters on respiratory physiology and technology. At this point, it’s necessary for me to point out that while this book is chock-full of great advice, none of it is specific to the care of any individual patient. Have any of your faculty ever told you that the patients don’t read the textbook? They’re right. Every patient needs an individualized approach. Believe it or not, my lawyer didn’t make me write this. It’s just common sense.
Philosophy of Mechanical Ventilation The art of medicine consists of amusing the patient while Nature takes its course. —Voltaire Mechanical ventilation is a wonderful tool. The birth of modern-day critical care occurred in Copenhagen in 1952, when Bjorn Ibsen realized that positive pressure ventilation could save lives during a polio epidemic when the iron lungs (a negative pressure ventilator) were failing. The most common reason for admission to a medical intensive care unit is the need for mechanical ventilatory support. The combination of endotracheal intubation and positive pressure ventilation has likely saved hundreds of thousands, if not millions, of lives. Likewise, artificial ventilation has prolonged the lives of thousands of people afflicted with spinal cord injuries and devastating neuromuscular diseases. Ventilators attached to wheelchairs permit patients with these conditions to engage in life, to pursue their interests, and to generally live lives that would not have been possible a half-century ago. Truly, this invention has had a positive effect on many, many people. As is the case with any technology, however, there is the potential for misuse. It is essential that anyone working in an intensive care unit remember the Third Commandment—that the ventilator is a means of support, and not a cure for any condition. In other words, it is folly to believe that the application of mechanical ventilation can reverse chronic lung disease, malignancy, congestive heart failure, or any of the myriad diseases and injuries that result in respiratory failure. The ventilator exists to maintain the respiratory and metabolic functions of the lungs until the patient recovers from his or her illness. It cannot make the patient better by itself. This is actually a point lost on many physicians, who believe that small tweaks and adjustments to the ventilator will accelerate the patient’s recovery from acute respiratory failure. If it is important for physicians to understand the natural history and
trajectory of a patient’s disease, it is equally important that the physician present this information to the patient and his family in concise, understandable, and even blunt terms. A life spent connected to a ventilator may be acceptable to a patient with amyotrophic lateralizing sclerosis, who may require mechanical ventilation but can otherwise speak, interact, and engage in what he considers an acceptable quality of life. It is a different matter entirely for a patient suffering from a massive intracerebral hemorrhage who is comatose, and is expected to remain comatose for, if not the rest of his life, a great deal of it. While the patient or his family may consider this to be a worthwhile existence, it behooves the physician to inform them of the stark realities of preserved life on a ventilator (including the medical, social, and financial ramifications) before they pursue this treatment option. So, what is a dedicated, caring physician, nurse, or respiratory therapist to do? Unsubstantiated optimism can be harmful, but so can overly pessimistic nihilism. Most patients with respiratory failure who recover from the inciting illness or injury will recover; true ventilator dependence, meaning a need for mechanical ventilation more than a year afterward, is rare. Here’s what we can do: 1. 2. 3. 4.
5. 6. 7.
Protect the lung from iatrogenic injury. Use an evidence- and physiology-based approach to ventilator settings. Promptly and aggressively treat the inciting illness or injury. No disease is effectively treated with starvation. Proper nutritional support is very important. People aren’t meant to lie in bed all day. Unless the patient is comatose, in shock, or has profound respiratory failure, it’s time to start getting him out of bed and into a chair. Walking, even. I’ll add that this, of course, requires a strong dose of common sense. Mobilizing a patient with an open sternum might not be a good idea. But it’s surprising how many patients lie flat on their backs for their entire ICU stay. Not healthy. When the patient seems to be recovering, start assessing his readiness for extubation every day. Be patient. It might take longer than you think. Once it’s evident that the patient will require prolonged mechanical ventilation, get on with the tracheostomy. There’s no need to wait an
8. 9. 10.
arbitrary number of days. Pay attention to the little things like DVT prophylaxis, skin care, and preventing delirium. Be patient. And…. Remember that your patient is a fellow human being with wants, needs, cares, and concerns that may be strikingly similar to your own. He deserves to be spoken to, even if he can’t speak back. He deserves respect, even though he may not be able to return that respect. He deserves the basics of human kindness and touch. Remember that he has placed his life in your hands. Your job is not an easy one, and not one that most people can do. The recognition that you have positively affected the life of another person in a way that few can is the greatest reward of this great profession.
Chapter 1
Initial Settings *Note
on measurements—unless otherwise specified, all airway pressures are measured in cm H2O. Gas pressures (PaO2, PaCO2) are measures in mm Hg. All tidal volumes are expressed as mL/kg of predicted body weight (PBW).
Modes of Ventilation There are several different modes of ventilation, and each ventilator manufacturer has its own (usually trademarked) name for them (PRVC, VC+, CMV with Autoflow, ASV, PAV, Volume Support, and the list goes on and on). This can be intimidating at first—who’s to know what to pick? Fortunately, like medications, all of these have a generic name as well. That’s all you really need to know, because all of the modes on the different ventilators available for sale will be essentially the same (just with a different trade name). Each mode of ventilation has its strengths and weaknesses. No mode is perfect, and no mode is useless. It’s best to pick the mode that best suits the patient’s needs at the time. Each of these modes is discussed in more detail in the following chapters, but here’s a brief overview.
Assist-Control Ventilation Assist-Control Ventilation is the mode of choice in most circumstances. It allows the ventilator to essentially take over the work of breathing and is preferred when a patient has acute cardiac or respiratory failure. It provides full respiratory support. If the patient wants to breathe over the set rate, he can; when he triggers the ventilator, he gets the full breath with minimal effort.
Upside: Takes over the work of breathing; clinician can choose to set a tidal volume (volume control) or an inspiratory pressure (pressure control). Downside: A tachypneic patient will get the full tidal volume on every breath, so without adequate sedation this could lead to significant respiratory alkalosis or air trapping. This can be a problem in patients with COPD or asthma.
SIMV with Pressure Support SIMV also can provide full ventilator support and is a very popular mode. Like Assist Control, the clinician can choose a tidal volume or an inspiratory pressure. The major difference between SIMV and Assist Control is what happens when the patient initiates a breath— in A/C, he gets the full tidal volume; in SIMV, he gets whatever he can pull (usually with the help of pressure support). Upside: Can take over the work of breathing but allows the patient more spontaneous breathing than in assist-control. Can be useful for weaning support gradually. Downside: If the machine rate is not set high enough, an unstable patient can get fatigued due to excessive work of breathing. If the pressure support is not set high enough, spontaneous breaths may be fast and shallow, which also leads to fatigue.
Pressure Support Ventilation PSV doesn’t have a set rate—instead, it allows the patient to breathe on his own and “boosts” each breath with a pressure that the clinician selects. It’s used in conjunction with CPAP to improve alveolar recruitment. PSV is used in patients who are either intubated for reasons other than cardiac or respiratory failure (altered mental status, jeopardized airway) or for weaning. It can also be used when the patient has a severe metabolic acidosis—if he has a pH of, say, 6.88 and a HCO3 of 4, his respiratory drive will be markedly elevated and a mode like assist-control may not be able to meet his metabolic demands. Upside: Allows the patient to set his own rate and pattern of breathing, which
is more comfortable; spontaneous breathing has salutary effects on hemodynamics and VQ matching. Downside: There’s no backup rate, so if the patient goes apneic nothing will happen until the alarms sound. Unstable patients will fatigue rapidly if the work of breathing is imposed on them, even with high levels of pressure support.
Unconventional Modes Airway pressure release ventilation (APRV) and high frequency oscillatory ventilation (HFOV) are used to treat severe hypoxemia. They are seldom the first-line option for acute respiratory failure and will be discussed later in the book. For now, just focus on the modes already listed (A/C, SIMV, PSV).
Ventilator Settings Based on Pathophysiology Restrictive Lung Disease Examples: ARDS, aspiration pneumonitis, pneumonia, pulmonary fibrosis, pulmonary edema, alveolar hemorrhage, chest trauma Restrictive lung diseases are associated with a reduction in respiratory system compliance. The lungs want to collapse. In other words, it’s hard to get air in and easy to get air out. The ventilation strategy is to recruit vulnerable alveoli, prevent cyclical alveolar closure, provide adequate oxygenation, and to minimize volutrauma from overdistension. The initial mode should be one that takes over the work of breathing for the patient. Assist-control, using either volume-controlled or pressure-controlled ventilation, is the mode of choice. For volume-controlled ventilation: 1. 2. 3. 4.
Tidal volume of 6 mL/kg PBW Rate of 14-18 breaths per minute, with a decelerating flow pattern FiO2 100% at first; reduce to 60% if SpO2 ≥ 88% PEEP of 5-10 cm H2O, depending on the degree of hypoxemia. Remember, the more opacification in the lungs on the chest X-ray, the
5. 6.
more PEEP will be needed to reduce intrapulmonary shunting. If hypoxemia persists, increase the PEEP until the SpO2 is 88% or better. Don’t exceed 20. After adjusting the PEEP, check the plateau pressure. If the PPLAT is more than 30 cm H2O, decrease the tidal volume until the PPLAT is less than 30. Don’t go below 4 mL/kg PBW.
For pressure-controlled ventilation: 1. 2. 3. 4. 5.
6. 7.
PEEP of 5-10 cm H2O, depending on the degree of hypoxemia FiO2 100%; reduce to 60% if SpO2 ≥ 88% Inspiratory pressure of 15-20 cm H2O Rate of 14-18 breaths per minute Inspiratory time adjusted to keep the I:E ratio 1:1.5 or higher. The Itime is usually 1.0-1.5 seconds. A rate of 20 and an I-time of 1.0 seconds has an I:E ratio of 1:2 (one second inspiration, two seconds expiration). A rate of 15 with an I-time of 1.5 seconds has an I:E ratio of 1:1.7 (1.5 seconds inspiration, 2.5 seconds expiration). This is displayed on the ventilator screen. If hypoxemia persists, increase the PEEP until the SpO2 is 88% or better. Don’t exceed 20 cm H2O. Look at the exhaled tidal volume. If it exceeds 6 mL/kg, lower the inspiratory pressure until the tidal volume is in the 4-6 mL/kg range.
After initiating ventilation, check an arterial blood gas. 15-20 minutes is enough time for gas exchange to equilibrate. Make changes in the respiratory rate to change the PaCO2 (a higher rate lowers the PaCO2, and vice versa). Leave the tidal volume in the 4-6 mL/kg range, keeping the PPLAT at 30 cm H2O or less. Remember that lung protection is more important than normal ventilation—a pH of 7.15 or better is acceptable and it’s not worth injuring the lungs with overdistension (in the form of high tidal volumes) to get a normal pH or PaCO2. Lower the FiO2, keeping the PaO2 between 55 and 70 mm Hg and the SpO2 between 88% and 94%. There’s nothing to gain from keeping the PaO2 above
this range, with few exceptions. Patients with traumatic brain injury sometimes require a higher PaO2, usually in conjunction with brain tissue oxygen monitoring. Victims of carbon monoxide poisoning also benefit from breathing 100% oxygen.
Obstructive Airways Disease Examples: COPD, Asthma Obstructive lung disease is associated with an increase in respiratory system compliance and an obstruction to expiratory airflow. It’s easy to get air in, but hard to get it out. The ventilation strategy is to rest the respiratory muscles, provide adequate oxygenation, and reduce hyperinflation. Assist-control ventilation is usually the mode of choice, and volume-control is preferable to pressure-control. SIMV with PS can also be used, however, as long as the rate and PS are set high enough to prevent tachypnea and fatigue. High airway resistance and high peak inspiratory pressures characterize exacerbations of COPD and asthma, even though the PPLAT may be significantly lower. Using pressure-control in this situation leads to very low tidal volumes. Volume-control guarantees that the desired tidal volume will be delivered. 1. 2. 3.
4.
Tidal volume of 8 mL/kg PBW. Lower tidal volumes can lead to air trapping and worsening hyperinflation. Rate of 10-14 breaths per minute Inspiratory time adjusted to keep an I:E ratio of 1:3 or higher. In obstructive airways disease, air gets in easily but has a hard time getting out due to narrow, inflamed bronchioles and bronchi. Give the air some time to escape. With asthma, applied PEEP will worsen hyperinflation. With COPD, PEEP can be used to splint open airways that are prone to collapse. This is because COPD is characterized by dynamic airway obstruction, while the obstruction is fixed in an asthma exacerbation. A good starting point for both is a PEEP of 0, or ZEEP—zero applied endexpiratory pressure.
5.
FiO2 of 100% to start; lower this to 60% as long as the SpO2 remains 88% or better.
Sometimes, patients with COPD or asthma will remain tachypneic despite adequate sedation. In assist-control, every patient-triggered breath delivers a full tidal volume, and this can lead to air trapping or severe respiratory alkalosis. If this is the case, switching the mode to SIMV may help.
Severe Metabolic Acidosis Examples: Salicylate poisoning, septic shock, toxic exposures, acute renal failure, diabetic ketoacidosis The normal response of the respiratory system in the setting of metabolic acidosis is to hyperventilate. CO2 is a volatile acid, and the lungs can rapidly eliminate this acid from the body in an attempt to bring the pH closer to normal. In a patient with a HCO3 of 4 mEq/L, for example, the PaCO2 will be 14-15 mm Hg if there’s appropriate respiratory compensation. This requires a very high minute ventilation to accomplish. It is very difficult to set the ventilator to provide a high minute ventilation, even if you set the rate to be 30-35 and the tidal volume to be 800-1000 mL. Patients with severe metabolic acidosis will often breathe in when the vent is trying to breathe out, and vice versa—this leads to significant patientventilator dyssynchrony and alarming of the machine. More consequentially, the volume and pressure alarms that are normally helpful will actually work against the patient by limiting the minute ventilation that can occur. Consider the aforementioned example—a patient who has a pH of 6.88 and a HCO3 of 4 needs a PaCO2 of 14-15. If he’s intubated and sedated, and the vent settings are put in the “usual” range, his PaCO2 may rise to 25-30. In the setting of severe acidemia, this increase in CO2 will cause his pH to fall to 6.6 or so, which will most likely lead to a cardiac arrest. The best way to deal with this situation is to let the patient’s naturally high respiratory drive work in his favor. 1.
Use the bare minimum of sedation to intubate and avoid neuromuscular
2. 3. 4. 5.
blockers entirely. Set the vent mode to be Pressure Support Ventilation. CPAP (a.k.a. PEEP) 5-10 cm H2O, depending on the degree of hypoxemia Pressure Support (PS) of 10-15 cm H2O. Adjust if needed to allow the patient to breathe comfortably; most of the time, 10 cm is enough PS. Allow the patient to have a high minute ventilation—it may be 18-25 L/min or higher. Don’t be alarmed to see him pull spontaneous volumes of 1000-2000 mL. The high minute ventilation will keep the pH up while the cause of the metabolic acidosis is being treated.
Key Concepts for Other Clinical Situations The left ventricle likes PEEP—increasing the intrathoracic pressure lowers preload and afterload, which is beneficial in acute cardiac failure due to left ventricular dysfunction (either systolic or diastolic). The right ventricle, on the other hand, doesn’t care for PEEP very much. Increased intrathoracic pressure can increase pulmonary vascular pressures and stress the thin-walled RV. In situations where RV failure is present (massive pulmonary embolism, worsening pulmonary hypertension), use more FiO2 and less PEEP (ideally 10 cm or less) to maintain oxygenation. When there is acute brain injury, be it from stroke, hemorrhage, trauma, or something else, the priority with mechanical ventilation is the maintenance of adequate oxygenation. Aim for an SpO2 of 94-98% and a PaO2 of 80100 mm Hg. PEEP may increase the intracranial pressure, but it seems to be significant only when the PEEP is 15 or higher. Hypoxemia, on the other hand, definitely increases intracranial pressure. Therefore, use what it takes to maintain adequate cerebral oxygenation. Hyperventilation (PaCO2 < 32) lowers intracranial pressure, but it works by causing cerebral vasoconstriction. In other words, it works by making the brain ischemic. This may be helpful if a patient is about to herniate, and you need 5 minutes to get the mannitol in or 10 minutes to get to the OR. Prolonged hyperventilation, on the other hand, worsens brain ischemia and has no lasting effect on intracranial hypertension. Aim for a normal (35-40) PaCO2. Chapter 2 Quick Adjustments and Troubleshooting Problem: Gas Exchange Abnormalities These are ways to adjust the ventilator based on the arterial blood gas. Obviously, the patient’s condition should dictate what’s done. The adjustments are in order of preference. PaO2 Too Low Assist-Control, SIMV: increase PEEP, increase FiO2 APRV: increase PHIGH, increase THIGH, increase FiO2 HFOV: increase mean airway pressure, increase FiO2 PaCO2 Too High Volume Assist-Control or SIMV: increase rate, increase tidal volume Pressure Assist-Control or SIMV: increase rate, increase driving pressure APRV: increase the gradient between PHIGH and PLOW, decrease THIGH, increase TLOW HFOV: decrease the frequency, increase the amplitude, increase TI%, allow a 5 cm cuff leak PaCO2 Too Low Volume Assist-Control or SIMV: decrease rate, lower tidal volume Pressure Assist-Control or SIMV: decrease rate, lower driving pressure APRV: increase THIGH, lower PHIGH, decrease TLOW HFOV: increase the frequency, lower the amplitude, decrease TI% These are problems that you’ll be called about. As always, the first thing you should do is examine the patient. Remember your ABCs and use this guide to help you figure out what’s wrong. Problem: High Peak Airway (PAW) Pressures Your first step should be to perform an inspiratory pause and measure the plateau pressure (PPLAT). The plateau pressure represents the alveolar pressure, while the peak pressure is a combination of the alveolar pressure and airway resistance. High PAW, Low PPLAT—this means the problem is high airway resistance. Kinked endotracheal tube—unkink the tube Mucus plugging—pass a suction catheter Bronchospasm—inhaled bronchodilators Too narrow of an endotracheal tube—change the tube, or accept higher PAW High PAW, High PPLAT—this means the problem is in the lungs. Mainstem intubation—pull the endotracheal tube back into the trachea Atelectasis of a lobe or lung—chest percussion, or bronchoscopy to open up the airway Pulmonary edema—diuretics or inotropes ARDS—use a lower tidal volume, higher PEEP strategy Pneumothorax—chest tube Problem: Dynamic Hyperinflation (Auto-PEEP) This is usually due to inadequate time for exhalation. High airway resistance (bronchospasm, COPD, mucus plugging) makes it worse. On exam, the patient’s abdominal muscles will contract forcefully during exhalation. Neck veins may be distended, and you may hear loud wheezing. The ventilator’s expiratory flow waveform will not return to the baseline of zero flow. Lower the ventilator rate, usually between 10-14 breaths per minute Shorten the inspiratory time to keep the I:E ratio in the 1:3 – 1:5 range Keep the tidal volume in the 6-8 mL/kg range—a higher tidal volume will often slow the patient’s spontaneous respirations Increase the inspiratory flow to 60-80 liters per minute if the patient seems to be “air hungry” Adequate sedation with narcotics will help blunt a tachypneic response Treat bronchospasm with inhaled bronchodilators and systemic steroids Problem: Sudden drop in SpO2 New or worsening hypoxemia is always cause for alarm. The first step is to exclude mechanical problems or tube displacement. Disconnect the patient from the ventilator and bag him Make sure the tube is in place (use either color-change or waveform capnometry if there’s any doubt about the tube) and that breath sounds are present and equal Obtain an arterial blood gas Chest X-ray—this will show you worsening infiltrates, pneumothorax, pulmonary edema, atelectasis, or new effusions Always consider pulmonary embolism as a cause for new hypoxemia in an ICU patient, and have a low threshold for diagnostic studies Absent breath sounds on one side—pull the endotracheal tube back a few centimeters Absent breath sounds on one side, even with the tube in the right place —think pneumothorax, or mucus plugging with complete atelectasis of the lung Tension pneumothorax should be suspected if breath sounds are absent on one side and if the patient is hypotensive. Distended neck veins and tracheal shift away from the affected side are supportive but not always seen. The treatment is immediate needle decompression and placement of a chest tube. Problem: Fighting the Ventilator Before sedating or paralyzing a patient for “fighting the ventilator,” you should always check TSS—Tube, Sounds, Sats. Make sure that the endotracheal tube is in place and not obstructed, that breath sounds are present and equal, and that the patient is not hypoxemic. Other things you should look for are: Dynamic hyperinflation (see above for how to treat this) Untreated pain, especially in trauma and surgical patients Make sure the vent is providing an adequate rate and tidal volume Switch to assist-control ventilation, if the patient is getting fatigued Search for other causes of distress—cardiac ischemia, fever, abdominal distension, neurologic deterioration, etc. Problem: Change in End-Tidal CO2 First, look at the waveform. If there is no waveform, it means one of three things: The endotracheal or tracheostomy tube is not in the trachea The tube is completely occluded The ETCO2 sensor is faulty Obviously, the first two are serious emergencies and should be dealt with immediately. The third is diagnosed only after ruling out the first two. If the waveform is present, then look at the ETCO2 value. With a significant change in the ETCO2, an arterial blood gas should be obtained as well to see what the PaCO2 is. Rising ETCO2 and PaCO2—this indicates either increased CO2 production or alveolar hypoventilation. Fever Malignant hyperthermia Thyrotoxicosis Suppressed respiratory drive without a sufficient ventilator backup rate Falling ETCO2 with unchanged or rising PaCO2—the widening gradient between the two suggests an increase in dead space ventilation. Pulmonary embolism Falling cardiac output (cardiogenic or hypovolemic shock) Dynamic hyperinflation with autoPEEP Falling ETCO2 and falling PaCO2—indicates an increase in alveolar ventilation. Pain Agitation Fever Sepsis Chapter 3 The Eleven Commandments of Mechanical Ventilation I. Thou shalt mind thy patient’s COMPLIANCE and measure it daily. Compliance is the change in volume divided by the change in pressure. Dynamic compliance is the exhaled tidal volume divided by the dynamic change in pressure (Peak minus PEEP). Static compliance is the exhaled tidal volume divided by the static pressure differential (Plateau minus PEEP). If there’s a big difference between the two, increased airway resistance is usually to blame. Normal respiratory system compliance is about 100 mL/cm H2O; normal for a ventilated patient is 70-80. Falling compliance can mean fluid overload, developing pneumonia or ARDS, pneumothorax, or many other bad things. Improving compliance usually means the patient is getting better, at least from a pulmonary mechanics point of view. II. ‘Tis nobler to INTUBATE and VENTILATE than to needlessly allow a patient to suffer the slings and arrows of critical illness. Intubating a critically ill patient is never a sign of weakness; rather, it is a sign of decisiveness. A few of the indications for intubation are refractory hypoxemia, hypercapnia, a jeopardized airway, shock, and great metabolic disturbances. III. Thy mechanical ventilator is merely a means of SUPPORT and offers no curative properties in itself. It’s a mistake to think that the ventilator itself can help the patient. It merely allows the patient to survive until he recovers. A ventilator has only three therapeutic benefits: 1) Guaranteed delivery of high levels of oxygen 2) Positive pressure to reduce intrapulmonary shunt (from atelectasis, ARDS, pneumonia, pulmonary edema, etc.) 3) Providing the work of breathing until the patient is able to do it himself IV. Thou shalt be familiar with the ABUNDANCE of MODES, as no one is perfect for every situation and no one is completely useless. While you may have your preferred mode, remember that you can ventilate most any patient with any given mode as long as you set the ventilator properly. Some patients will seem to prefer one mode over another. I don’t know why this happens, but it does. Deal with it and don’t be afraid to find out what vent settings suit the patient best. V. Thou shalt mind the TIDAL VOLUME closely and without fail, lest the lungs suffer from excessive distension. Of all the studies done on mechanical ventilation in acute lung injury and ARDS, the only thing that seems to affect survival is the use of excessive tidal volumes. Your resting tidal volume is 4-8 mL/kg of your predicted body weight. Your patient’s should be as well. The patient’s actual body weight should not be used for this calculation. Carry a table, memorize the formula, or download an app to figure out the PBW. You will need the patient’s height and gender (both usually easy to obtain). Be wary of physicians who confidently tell you that the plateau pressure is more important, or that 7, or 8, or 9 mL/kg is better than 6 in ARDS—while they may be right, they possess no evidence to support their claims. VI. Thou shalt OPEN thy patient’s lungs and KEEP THEM OPEN. Positive end-expiratory pressure is used to recruit collapsed alveoli and to prevent them from closing during exhalation. This helps to restore at least some functional residual capacity and reduce intrapulmonary shunt. A general rule is that if you can see white stuff in the lungs on the chest X-ray, increasing the PEEP is better than using high levels of oxygen for correcting hypoxemia. VII. For the PERFECT ABG is a mythical creature and should not be pursued lest the patient suffer grave harm in the form of barotrauma and volutrauma. It’s more important to protect the patient from harm than to blindly pursue a “normal” blood gas, especially if it means using excessively high tidal volumes or ventilator pressures. All decisions regarding ventilator settings should be made with the whole patient in mind. Permissive hypercapnia is perfectly acceptable in status asthmaticus but not in the patient with cerebral edema. In most cases, a PaO2 of 55 is adequate. VIII. Thou shalt not allow thy shocked patient to FATIGUE, but instead provide the ventilator support necessary for him to recover. In the setting of shock, hemorrhage, or severe sepsis, work of breathing can account for 40-50% of a patient’s basal energy expenditure. Mechanical ventilation should be used to take over this work until the underlying cause has been treated adequately. Assist-control ventilation is one of the best ways to do this and is the preferred mode most of the time in these situations. There are many theories about exercising the diaphragm and allowing the patient to “work out” on SIMV or CPAP/PSV, but no one has proven that it helps (and it may in fact be harmful). Assist-control, with a daily spontaneous breathing trial if indicated, is a simple formula that is also very effective at minimizing the time a patient stays on the ventilator. IX. Thou shalt seek out DYNAMIC HYPERINFLATION wherever it may be found, and treat it, for ‘tis an insidious beast! Hyperinflation is also known as auto-PEEP or breath stacking. It occurs when the patient can’t get all of the air out before the next breath starts. If unchecked, dynamic hyperinflation can lead to discomfort, hypercapnia, hypotension, and even PEA arrest. Suspect it in all patients on the vent who have obstructive lung disease and look for it even in patients who don’t. If the PaCO2 keeps going up as the rate is increased, hyperinflation is the likely culprit. Treat this condition by slowing the ventilator rate, extending the time for exhalation, and treating bronchospasm. A small measure of applied PEEP may help prevent airway collapse during exhalation. X. Verily, a SPONTANEOUS BREATHING TRIAL should be performed readily and daily on all patients whose conditions permit. No one is good enough to reliably predict which patients can be extubated on a given day. A spontaneous breathing trial (SBT) should be done whenever the reason for intubation (severe hypoxemia, coma, shock, bronchospasm) has resolved. The SBT can be in the form of a T-piece or low-level pressure support ventilation. Don’t be afraid to act on the results of the trial. If the patient looks ready, extubate him. The occasional reintubation is not a sign of failure. In fact, if you never reintubate a patient, you’re probably waiting too long to extubate the others. XI. Thy Respiratory Therapist is the ordained KEEPER OF THE VENT and should be treated with utmost esteem. Do not make any changes to the ventilator settings without the RT present. If you want to experiment with different settings to see what happens, call the RT first. While you may know what you’re doing, you probably don’t know how to reset all the alarms, sensors, etc. that have to be adjusted when significant changes are made. It’s also the RT’s responsibility, and if you make changes without notifying him it makes a difficult job even harder. Chapter 4 Acute Respiratory Failure Acute respiratory failure is one of the most common reasons for admission to the intensive care unit. The majority of cases will require some sort of positive pressure ventilation, either from a mask (CPAP, BiPAP) or an endotracheal tube. Obviously, this is important. There’s a reason why A and B come first in the ABCs of resuscitation—without adequate gas exchange (oxygenation being the most important), the patient can die within minutes. Many times, the physician has to treat acute respiratory failure before he can figure out the whys and hows of what happened. That’s OK! Once the patient is stabilized, however, the detective work begins. Acute respiratory failure, according to the textbook by Parrillo and Dellinger, is “the inability of the respiratory system to meet the oxygenation, ventilation, or metabolic requirements of the patient.”1 Let’s break this definition down: “The respiratory system”: More than the lungs. Obviously, the lungs are the major players, but disorders of the upper airway, chest wall, cardiovascular system, and neurologic system can cause significant respiratory dysfunction. “Oxygenation requirements”: Type I respiratory failure is defined as a PaO2 less than 60 mm Hg. The first priority in treating patients with acute respiratory failure is to correct hypoxemia! “Ventilation requirements”: Type II respiratory failure is a PaCO2 greater than 50 mm Hg, with a pH less than 7.30. The pH is important in distinguishing acute from chronic respiratory failure. “Metabolic requirements”: This is often forgotten, but the lungs have a key role in maintaining metabolic homeostasis. CO2 clearance by the respiratory system is adjusted to balance out metabolic derangements. Likewise, oxygen intake and delivery to the tissues begins in the lungs. “Of the patient”: Probably the most important part of the definition. A particular patient may have “normal” blood gas numbers but still require respiratory support. Conversely, another patient may have terrible numbers but not require any kind of acute intervention. Like everything else in medicine, start with the history and physical exam.* Common Diagnostic Testing Arterial Blood Gas: to determine whether or not respiratory failure is present, assess the metabolic status of the patient, and to determine (in part) the cause of respiratory failure. Co-oximetry can help diagnose carbon monoxide poisoning and methemoglobinemia. Chest X-ray: to diagnose cardiac failure, pneumonia, pneumothorax, pleural effusion, and a whole lot of other diseases. Also, helpful if it’s normal—a clear X-ray and hypoxemia should make you consider a pulmonary embolism. CT Chest: for a better look at the thoracic structures; if done with angiographic technique, it can diagnose pulmonary embolism and aortic dissection. Bronchoscopy: to diagnose inhalational injury, foreign body, upper airway obstruction, pneumonia, and alveolar hemorrhage. Hypoxemic Respiratory Failure Hypoxemia poses the most immediate threat to the patient. Vital organs like the brain, heart, etc. depend on a continuous delivery of oxygen to use for energy production. That’s why just about all resuscitation efforts begin with giving the patient supplemental oxygen (by nasal cannula, mask, or endotracheal tube). The pathophysiologic causes of hypoxemia are: 1. 2. 3. 4. 5. 6. 7. Shunt Ventilation-Perfusion (VQ) Mismatch Diffusion limitation Dead Space Low FiO2 (fraction of inspired oxygen) Low PB (barometric pressure) Alveolar hypoventilation Of the things in this list, low FiO2 and low PB are the easiest to rule in or out. Low FiO2 is seen in house fires (from consumption of oxygen by the flames) and anesthetic gas mishaps. Low PB includes things like airplane cabin depressurization and being at extreme altitude. Look around--if you’re not in an airplane, the building isn’t on fire, and you’re not on top of a mountain, these can be discarded from the differential diagnosis. Good! That leaves us with five things to worry about instead of seven. Diffusion limitation is really significant in only a few diseases like pulmonary alveolar proteinosis—most of the time the problem is VQ mismatch. Down to four. Sorry to introduce math into a clinical discussion, but it’s inevitable. If you want to do the job you must have the tools, and one important tool in this field is the Alveolar Gas Equation. This equation predicts what the alveolar pressure of oxygen should be. Alveolar Gas Equation where PAO2 is the partial pressure of oxygen in the alveolus, PB is barometric pressure (760 mm Hg at sea level), PH2O is the partial pressure of water in humidified air (47 mm Hg), and the RQ is the ratio of CO2 production to oxygen consumption. The RQ in most people is 0.8. Simplified for a patient breathing room air (FiO2 of 21%), the equation reads For a normal PaCO2 of 40 mm Hg, the PAO2 should be 102 mm Hg. Normally, there is a small difference between the partial pressure of oxygen in the alveoli and that seen in arterial blood (the PaO2). This is called the A-a gradient, and it represents the small fraction of blood that doesn’t participate in gas exchange because of bronchopulmonary anastomoses. The normal gradient is less than 10 mm Hg, but this increases with age. It can also increase with supplemental oxygen. The predicted A-a gradient while breathing 100% oxygen can be as high as 110 mm Hg. Why is this important? Well, go back to your basic chemistry. The partial pressure of all of the gases in the alveolar space can only add up to the total pressure of the air. Therefore, if the PaCO2 is elevated, the PAO2 has to go down. Only so many marbles in the jar, so to speak. If the PAO2 goes down, the PaO2 falls as well. Hypoxemia can be due solely to hypercarbia if the PaCO2 gets high enough, and a normal A-a gradient means that that there is no problem with the lungs or the pulmonary circulation. The hypoxemia is a result of inadequate ventilation alone (pure Type II respiratory failure). If the A-a gradient is widened, then there must be some venous admixture. The three mechanisms of venous admixture are shunt, dead space, and VQ mismatch. Shunt Shunt is easy to visualize—blood passing from the right side of the heart to the left side of the heart through areas where there is no ventilation whatsoever. The V/Q ratio is zero. A shunt can be intracardiac or intrapulmonary. The gas exchange abnormality with shunt is profound hypoxemia with preserved ventilation. The PaCO2 doesn’t begin to rise until the shunt fraction exceeds 40-50% of the pulmonary blood flow. The normal shunt fraction is less than 3%. Intracardiac shunts in adults include uncorrected atrial septal defects and ventricular septal defects. The Eisenmenger Syndrome is a VSD with shunt reversal (it begins left-to-right, but as the right ventricle hypertrophies the shunt becomes a right-to-left one and the patient becomes hypoxemic). Pulmonary shunts are caused by something preventing inspired gas from reaching alveoli. Examples include atelectasis, ARDS, pulmonary edema, and consolidation from pneumonia. Shunt is characterized by hypoxemia resistant to correction. As the shunt fraction increases, the hypoxemia will get worse despite breathing high levels of oxygen. With a shunt fraction of 50%, even the administration of 100% oxygen will rarely take the PaO2 above 60 mm Hg.2 Therefore, the treatment of hypoxemic respiratory failure resulting from a shunt requires more than supplemental oxygen. Positive pressure ventilation to recruit and stabilize collapsed lung units is required. The shunt fraction can be calculated if the mixed venous oxygen content can be sampled using a pulmonary artery catheter (see Appendix for the equation). This has to be done with the patient breathing 100% oxygen to eliminate the contribution of VQ mismatch to the hypoxemia. Because the equation is cumbersome and requires invasive testing, it’s useful to have a shortcut. The P/F ratio is calculated by dividing the PaO2 by the FiO2. For example, say a patient has a PaO2 of 100 mm Hg on 60% oxygen. His P/F ratio is 100/0.6, or 167. A P/F ratio less than 200 suggests a shunt fraction greater than 20%. VQ Mismatch Normal cardiac output is 5 L/min. Normal minute ventilation (rate × tidal volume) is 4 L/min. The average ventilation-perfusion ratio, then, is 4/5 or 0.8. As the metabolic demands of the body increase, cardiac output and minute ventilation increase accordingly. Areas that are poorly ventilated don’t get much blood flow, though, because of hypoxic pulmonary vasoconstriction. In every other organ system, hypoxia results in vasodilatation. In the lungs, however, alveolar hypoxia causes vasoconstriction. This is a good thing—why send blood to lung units that don’t have a whole lot of oxygen to drop off to the red blood cells? When this balance is lost, VQ mismatch occurs and leads to hypoxemia. This is also the reason why patients with COPD and chronic CO2 retention get a respiratory acidosis when someone gives them high levels of oxygen. Certain disease states can alter airway caliber and tone and affect gas delivery to the alveoli, which results in lung units which are more perfused than ventilated—a VQ ratio less than 0.8. Examples of this are asthma, COPD, interstitial lung disease, tracheobronchitis, and pneumonitis. Other diseases may impede proper blood flow to ventilated units, causing more ventilation than perfusion and a VQ ratio of more than 0.8. This can be seen with chronic thromboembolic disease, vasculitis, and overdistension of alveoli during positive pressure ventilation. Patients who are supine and are on a ventilator have VQ mismatch owing to the air going anterior and the blood going posterior due to gravity. VQ mismatch is the most common cause of hypoxemic respiratory failure and usually corrects readily with supplemental oxygen. Even severe cases of VQ mismatch are responsive to breathing 100% oxygen. Inability to correct the PaO2 with high levels of oxygen suggests a shunt. Dead Space Ventilation Dead space is the opposite of shunt—the alveoli are ventilated but there is absolutely no perfusion. The VQ ratio is ∞. Dead space is seen with large pulmonary embolism, venous air embolism, and low cardiac output. It can also be seen with significant overdistension of alveoli during positive pressure ventilation and dynamic hyperinflation in patients with COPD. The gas exchange abnormality seen with dead space ventilation is both hypoxemia and hypercarbia. The CO2 is not cleared because the venous blood never comes in contact with alveoli. Remember that everyone has anatomic dead space, which refers to the trachea and large airways that hold air but don’t participate in gas exchange. This is usually 150-180 mL, about 1 mL for every cm in height. This volume is part of the minute ventilation and should account for less than 30% of the tidal volume (VD/VT ≤ 0.3). Rapid shallow breathing can increase the proportion of the minute volume that is dead space. For example, a patient with a tidal volume of 500 mL has an anatomic dead space of 150 mL and a VD/VT ratio of 0.3. His respiratory rate is 12, for a minute volume of 6 L/min (of which 1.8 L is wasted dead-space ventilation and 4.2 L is alveolar ventilation). If his respiratory rate increases to 30 and his tidal volume drops to 200 mL, he still has the same minute volume of 6 L/min. His dead space is still 150 mL per breath, though, which means that 4.5 L/min (30 × 150 mL) of his minute ventilation is wasted, leaving only 1.5 L/min for alveolar ventilation. His VD/VT ratio is now 0.75. In this case, the PaCO2 would rise and the PaO2 would fall due to alveolar hypoventilation. Tachypnea and labored respirations with a normal PaCO2 usually indicate increased dead space and a relative alveolar hypoventilation. This is one of the earliest signs of impending respiratory failure. It is nearly impossible to determine the PaCO2 with any kind of accuracy by clinical exam, so arterial blood gas measurements are essential. Hypoxemia vs. Hypoxia Hypoxemia refers to a PaO2 less than 60 mm Hg. Hypoxia refers to inadequate delivery of oxygen to tissues or ineffective cellular utilization of oxygen, leading to anaerobic metabolism. It’s possible to be hypoxemic but not hypoxic, and it’s also possible to be hypoxic but not hypoxemic. Of course, you can be hypoxemic and hypoxic, or neither hypoxic nor hypoxemic. Clear enough? To better understand this, we have to consider how oxygen is delivered to the tissues. Oxygen is bound to hemoglobin and carried to the capillary beds, where it is unloaded into the cellular milieu; the deoxygenated hemoglobin then picks up CO2 and takes it back to the lungs to be excreted. Since 97% of the oxygen in the bloodstream is bound to hemoglobin, it makes more sense to focus on the percentage of hemoglobin saturated with oxygen than the partial pressure of oxygen in plasma. Oxygen Content Equation where CaO2 is the content of oxygen in arterial blood, expressed in mL O2/dL blood; Hgb is in g/dL; SaO2 is the arterial saturation of oxygen; and PaO2 is the partial pressure of oxygen in plasma. For a normal person with hemoglobin of 15 g/dL, SaO2 of 100%, and PaO2 of 100 mm Hg, the CaO2 is 20.4 mL O2/dL blood. The oxygen dissolved in plasma contributes 0.3 mL O2, or less than 1.5% of the total. Oxygen delivery is the CaO2 multiplied by the cardiac output. It’s necessary to multiply this by 10, since CaO2 is measured in deciliters and cardiac output is measured in liters. A normal person with a cardiac output of 5 L/min has an oxygen delivery (DO2) of 1020 mL O2/min. Looking at the above equations, you can see that the most important factors governing oxygen delivery are the cardiac output, hemoglobin content, and the arterial oxygen saturation. The PaO2 plays a minor role. Four Types of Hypoxia Hypoxemic: low SaO2 leading to low delivery of O2 to the tissues. Stagnant: low cardiac output, which leads to tissue hypoxia even if the patient is breathing 100% oxygen. Anemic: not enough red blood cells to carry oxygen to the tissues. Cytopathic: the heart pumps enough oxygen to the tissues, but something inhibits effective oxidative phosphorylation (septic shock, cyanide poisoning, salicylate poisoning). Hypercarbic Respiratory Failure Type II, or hypercarbic*, respiratory failure is due to an inability of the body to clear CO2. Hypoxemia may occur due to hypoventilation, but this is correctable with supplementary oxygen. The best way to determine the cause of hypercarbic respiratory failure is to consider the various ways the body controls CO2 elimination and look for breakdowns in the system. Normally, the body is very good at maintaining a normal PaCO2. Even during deep sleep, the PaCO2 varies by 2-3 mm Hg at most. This balance is maintained by the respiratory centers in the medulla oblongata, which stimulate diaphragmatic contractions via the phrenic nerve. When acute hypercarbic respiratory failure occurs, the problem can be localized to either the signaling pathways of the nervous system or the bellows of the respiratory system. Ask yourself—where is the problem? Localizing the Cause of Hypercarbia Brainstem: drug overdose, trauma, intracerebral or subarachnoid hemorrhage, infection, obesity hypoventilation syndrome, hepatic or uremic encephalopathy, bulbar poliomyelitis? Spinal Cord: lesion at C4 or higher, central cord hematoma, traumatic injury, “high spinal” anesthesia, polio, epidural abscess, transverse myelitis? Peripheral Nerves: phrenic nerve paralysis, tick paralysis, acute inflammatory demyelinating polyneuropathy (Guillain-Barré syndrome), acute intermittent porphyria, heavy metal poisoning? Neuromuscular Junction: botulism, myasthenia gravis, paraneoplastic syndrome, neuromuscular blocking drugs? Muscles: muscular dystrophy, dermatomyositis, hypothyroidism, hypophosphatemia, hypermagnesemia, myopathy, critical illness polymyopathy? polymyositis, hypokalemia, steroid-induced Thoracic Cage: kyphoscoliosis, thoracoplasty, morbid obesity, flail chest, abdominal compartment syndrome, ankylosing spondylitis, circumferential burn? Lungs: COPD? Dynamic hyperinflation? Upper Airway Obstruction Obstruction of the upper airway can cause respiratory failure. Common causes of upper airway obstruction requiring acute treatment are trauma, infection (peritonsillar abscess, retropharyngeal abscess), inhaled foreign body, inhalational injury, and angioedema. Stridor is a sign of airflow obstruction at or above the glottis, while expiratory wheezing usually indicates obstruction of the lower airways. Intubation should always be considered, preferably before the patient makes it clear that his airway is completely obstructed. Metabolic Control CO2 is the major byproduct of cellular metabolism. While hypercarbia is most often due to one of the disorders of ventilatory control, occasionally CO2 production can exceed the capacity of the respiratory system. This can occur during thyroid storm, malignant hyperthermia, cyanide or salicylate poisoning, and with massive catabolism. Ventilatory support may be needed. Hemodynamic instability and shock can also lead to respiratory failure due to the hypermetabolism seen with sepsis, trauma, and burn injuries. Blood that is directed toward the diaphragm and accessory muscles is shunted away from the splanchnic and hepatic circulation, which can lead to lactic acidosis. Excessive work of breathing can also worsen myocardial ischemia. A worsening metabolic acidosis or cardiac ischemia in the setting of shock are signs of impending respiratory failure and justify mechanical ventilatory support. A crucial part of managing acute respiratory failure in a patient with a severe metabolic acidosis is to provide enough minute ventilation to compensate for the metabolic process. If a man with ischemic bowel has bicarbonate of 4 and requires intubation, the minute ventilation should be set high enough to obtain a PaCO2 of 14 mm Hg, the expected amount of respiratory compensation. If the ventilator is set so that the PaCO2 is in the “normal” range of 35-45, the pH will plummet, and the patient could arrest. Treatment of Acute Respiratory Failure The most important thing is to find and treat the underlying cause, if possible. Support can be given to the patient during the workup and treatment and should not be delayed. Noninvasive support measures include supplemental oxygen and inhaled bronchodilators. Positive pressure ventilation can be given via a noninvasive mask system like CPAP or BiPAP —these are particularly effective for COPD exacerbations with hypercarbia and cardiogenic pulmonary edema. More severe manifestations of respiratory failure like ARDS, multilobar pneumonia, neuromuscular diseases, and cardiogenic shock usually require intubation and mechanical ventilation. With the exception of reducing the shunt fraction with positive end expiratory pressure, mechanical ventilation is not a therapeutic intervention. The goal of ventilation is to simply maintain adequate gas exchange and metabolic function while the underlying disease process either gets treated or (more commonly) resolves on its own. Thus, the physician should focus on treating reversible causes of respiratory failure and minimizing further injury to the patient. When the patient is ready to come off the ventilator, he’ll let you know. * In the future, patients can be plugged into a machine that will immediately analyze all of their medical problems and print out a list for the physician. I saw that on Star Trek. Until then, we still have to do an H&P. * Alternatively called hypercapnic Chapter 5 Oxygen Delivery and Consumption Many textbooks on respiratory and critical care medicine begin with statements like, “Oxygen is the most necessary and basic building block of life.” In clinical training, the early application of high-flow oxygen is taught as a life-saving maneuver in emergencies. In the emergency department and intensive care unit, much importance is placed on keeping the pulse oximeter reading over 90% (and usually over 95%); likewise, there is a compulsion to keep the PaO2 in the normal range of 90-100 mm Hg. At first glance, there is nothing wrong with this approach. Oxygen is indeed necessary for life, and avoiding hypoxemia is a core part of resuscitation. When treating patients with severe respiratory failure, however, attaining a normal PaO2 may be either impossible, or only possible by the application of injurious airway pressures. Therefore, a more complete understanding of oxygen delivery and consumption is necessary. Oxygen Content Each gram of hemoglobin can bind 1.34 mL of oxygen when fully saturated. A small amount of oxygen is also carried in the plasma in its dissolved form. This is represented by the PaO2. The solubility coefficient for oxygen in plasma is 0.003. Putting all of this together yields the oxygen content equation: With normal hemoglobin of 15 g/dL, SaO2 of 100%, and a PaO2 of 100 mm Hg, the oxygen content of arterial blood is 20.4 mL O2/dL blood. It is important to note that the contribution made by the dissolved oxygen (PaO2 x 0.003) is very small—0.3 mL O2/dL blood. The hemoglobin binds 98.5% of the oxygen content. The fraction contributed by the dissolved oxygen is negligible. If the FiO2 on the ventilator were increased to bring the PaO2 up to 500 mm Hg (keeping the SaO2 at 100%), only 1.2 mL O2/dL blood would be added to the oxygen content. Keeping the PaO2 elevated beyond what’s necessary for adequate saturation of the hemoglobin is unlikely to be consequential except in cases of profound anemia (Hgb < 5 g/dL) or hyperbaric conditions. In fact, the PaO2 can often be ignored when calculating oxygen content and delivery in order to make the math easier. This leads us to the first rule of oxygen: the SaO2 is what matters, not the PaO2. Oxygen Delivery Once the arterial blood is loaded with oxygen, it is delivered to the tissues to be used for metabolism. The amount of blood circulated per minute is the cardiac output, which is expressed in liters blood per minute. Since the CaO2 is measured in deciliters, the units are converted by multiplying by 10. This yields the oxygen delivery equation: If a normal cardiac output is 5 L/min, the DO2 is 1020 mL O2/minute. In order to make comparisons among different patients of various heights and weights, this can be indexed by dividing the DO2 by the body surface area. A “typical” body surface area is 1.7 m2, so the “typical” DO2I would be 1020/1.7, or 600 mL O2/min/m2. The cardiac output has the greatest influence on oxygen delivery. Even during periods of arterial hypoxemia, an increase in cardiac output can be sufficient to deliver the necessary amount of oxygen to the tissues. The table below shows the effect that an increase in cardiac output can have on oxygen delivery, even with significant anemia or hypoxemia. It also shows that anemia has a more pronounced effect on oxygen delivery than hypoxemia. For the purposes of simplifying the calculations, the PaO2 has been omitted. This leads us to the second rule of oxygen: an increase in cardiac output can offset hypoxemia. Effect of Different Variables on Oxygen Delivery CO Hgb SaO2 DO2 5 L/min 15 g/dL 100%1005 8 L/min 7 g/dL 100%750 8 L/min 15 g/dL 75% 1206 3 L/min 15 g/dL 100%603 Oxygen Consumption During periods of rest, the body’s consumption of oxygen (VO2) is approximately 200-250 mL O2/minute. Indexed for body surface area, the resting VO2I is 120-150 mL O2/min/m2. Normal subjects can increase their VO2 during peak exercise by a factor of 10, and elite athletes can reach a maximum VO2 of 20-25 times their resting consumption. During critical illnesses like septic shock, multisystem trauma, or burn injury, VO2 increases over baseline by approximately 30-50%. The consumption of oxygen by the tissues (VO2) varies by organ system. The brain and heart consume the most delivered oxygen, while hair, bones, and nails consume a negligible amount. This can be further complicated by the fact that different organ systems receive different amounts of the cardiac output—the brain consumes the most oxygen, for example, but also receives 15% of the total blood flow. The coronary circulation, on the other hand, accounts for only 5% of the total cardiac output so the percentage of delivered oxygen that is consumed is much higher. Fortunately for the clinician, this is not important because regional monitoring of oxygen delivery and consumption is practical only in laboratory animals. Measurement of the total body VO2, on the other hand, can be done rather easily with a pulmonary artery catheter (more accurate) or by using a combination of a noninvasive cardiac output monitor along with a measurement of central venous oxygen saturation (less accurate). While this is not as precise as directly measuring the content of oxygen in expired gas, it is a close enough approximation for clinical use. By measuring the mixed venous oxygen saturation in the pulmonary artery, the venous oxygen content can be calculated: As with the arterial oxygen content equation, the minor contribution made by the dissolved oxygen (in this case, the PvO2), can be omitted from the calculation. Thus, for a hemoglobin of 15 g/dL and a normal SvO2 of 75%, the venous oxygen content is 15.1 mL O2/dL blood. The difference between arterial and venous oxygen content is normally 3-5 mL O2/dL blood. The VO2 can then be calculated by multiplying the arterial-venous oxygen difference by the cardiac output and converting units: Expanded, this equation is: Rearranged (and simpler): In this case, with a cardiac output of 5 L/min, the DO2 is 250 mL O2/minute. Indexed for a typical body surface area of 1.7 m2, the DO2I is 147 mL O2/min/m2. Using the DO2 and VO2 Together Knowing the DO2 or VO2 in isolation is not particularly useful. The clinical question is whether the delivery is adequate to meet the body’s consumption requirements. To answer this, the DO2:VO2 ratio is helpful. During periods of both rest and exercise, the DO2:VO2 ratio is maintained at approximately 4:1 to 5:1 by changes in the cardiac output. This provides a reserve of sorts— after all, it wouldn’t be very useful from a survival perspective to only deliver as much oxygen as the body absolutely needs at any given time. This lack of a physiologic reserve would mean that a person would have no ability to withstand a sudden change in circumstances like having to sprint away from an attacker, or deal with a high fever or pulmonary embolism. As seen in the following figure, the DO2 can vary widely as the VO2 remains constant. This reflects the aforementioned physiologic reserve. As the DO2 declines, however, it can reach a point at which further drops in oxygen delivery cause a fall in consumption. This point is known in physiology as the hypoxic, or anaerobic, threshold. It is at this point that the reserve is exhausted, and the consumption becomes supply dependent. A patient at or below this point for any kind of prolonged period will become severely acidotic and, in most cases, will not survive. It would make sense that the anaerobic threshold would occur when the DO2 equals the VO2. Experimentally, however, it has been shown that the threshold is closer to the 2:1 mark and is explained by the variable oxygen consumption of different organ systems. Cardiac output delivered to hair, teeth, and bones doesn’t contribute much to meet the needs of the more vital organ systems. Mathematically, the DO2:VO2 ratio looks like this: Cancelling common factors greatly simplifies the equation: If the SaO2 is assumed to be 100%, then the SvO2 correlates with the DO2:VO2 ratio: DOv2:VO2 SvO2 5:1 4:1 80% 75% 3:1 2:1 67% 50% This correlation makes clinical estimation of the DO2:VO2 relationship much easier, as the SvO2 can be measured directly and continuously by a pulmonary artery catheter. If a pulmonary artery catheter is not present, a central venous oxygen saturation (ScvO2) can be measured by obtaining a venous blood gas from a central venous line placed in the internal jugular or subclavian vein. The ScvO2 is usually 5-8% higher than the SvO2. While not as accurate as the true mixed venous oxygen saturation obtained with a pulmonary artery catheter, the ScvO2 can be used to estimate of the DO2:VO2 relationship. The SvO2, as a surrogate for the DO2:VO2 relationship, can be used to identify when a patient has insufficient oxygen delivery to meet consumption requirements. The SvO2 also has the advantage of not requiring continuous calculation of the actual DO2 and VO2— any changes in the relationship between delivery and consumption will be reflected in the SvO2. The SvO2 drops as oxygen delivery drops relative to consumption. An SvO2 below 70% should warrant evaluation, and an SvO2 below 60% is definitely concerning —it means that the patient is approaching the anaerobic threshold. Looking back at the DO2 equation, impaired oxygen delivery is always due to either low cardiac output, anemia, or hypoxemia. Correction of these should increase DO2, with a resultant increase in SvO2. Keep in mind that the cardiac output has the most significant effect on DO2, and conditions like congestive heart failure, hypovolemia, hemorrhagic shock, and cardiac tamponade will all reduce cardiac output. This leads us to the third rule of oxygen: the SvO2 is low in low-flow states. Patients with severe respiratory failure may have uncorrectable hypoxemia. A reduction in the SaO2 will lead to a corresponding reduction in SvO2 if the DO2:VO2 ratio remains constant. Calculating the oxygen extraction ratio is a quick way to estimate the balance between oxygen delivery and consumption even when the SaO2 is markedly reduced: For a normal SaO2 of 100% and SvO2 of 75%, the O2ER is: (1.0– 0.75)/1.0 = 0.25/1.0 = 0.25, or 25%. This means that of the delivered oxygen, 25% was extracted and consumed by the tissues. A normal O2ER is 20-25%. As an example, consider a patient with severe respiratory failure whose SaO2 is 84%. His SvO2 is 60%. According to the above figure, an SvO2 this low would be concerning. However, the assumption in Figure 2 is that the SaO2 is 100%. Calculating the oxygen extraction ratio: While this is a bit higher than the normal range of 20-25%, it isn’t that much. Put another way, this indexing of the oxygen extraction would correlate with an SvO2 of 71.4% (if the SaO2 were 100%). As a second example, take a patient with severe respiratory failure with an SaO2 of 86%. His SvO2 is 49%. The O2ER is (0.86 – 0.49)/0.86, or 43%. This would correlate with an SvO2 of 57% if the SaO2 were 100% and is certainly concerning for a low cardiac output state. An O2ER of 30% or higher should warrant investigation, and an O2ER higher than 40% indicates that the patient is approaching the anaerobic threshold. The fourth rule of oxygen: the DO2:VO2 ratio, SvO2, and O2ER reflect the balance between delivery and consumption. They don’t represent a specific target for intervention. So, How Much Oxygen Is Really Needed? Unfortunately for physiologists and writers of clinical algorithms, simply saying to keep the SvO2 over 70% and all will be well doesn’t work. This should come as no surprise to anyone familiar with the medical literature in critical care medicine— multiple studies proposing one physiologic manipulation, or another have been consistently disproven. The combined processes of oxygen delivery, oxygen consumption, stress response, and cellular adaptation are far too complex to be summed up in this chapter, let alone a one-size-fits-all algorithm. A normal PaO2 while breathing ambient air at sea level is 90100 mm Hg, but humans are able to tolerate much less over prolonged periods of time. The minimum necessary PaO2 and SaO2 is not known, and it is unlikely that any IRB will grant approval to a study aiming to withhold supplemental oxygen from critically ill patients. The degree of tolerable hypoxemia is also highly variable, and depends on factors such as the patient’s age, comorbid conditions, living environment, genetic factors, and ability to cope with physiologic stress. What is known is that some people are able to survive moderate and even severe hypoxemia. Keep the following in mind: Mitochondrial PO2 in cardiac and skeletal muscle is normally between 1 and 5 mm Hg. Oxidative phosphorylation in mitochondria doesn’t begin to fail until the PO2 is between 0.1 and 1 mm Hg. Climbers on Mount Everest who obtained femoral arterial samples from each other had PaO2 in the 24-28 mm Hg range and lived to tell the tale. In septic shock, the problem is not inadequate oxygen delivery. It’s the inability of the tissues to properly metabolize the delivered oxygen. That’s why patients die despite having an SvO2 of 80%. The reasons for this are (very) incompletely understood. In the various ARDSNet trials, a PaO2 as low as 55 mm Hg (with an SaO2 of 88%) was considered acceptable. This is probably the best we will get as far as prospective evidence on the subject. Patients in the ARDSNet trial who received higher tidal volumes had better oxygenation, but also had a higher mortality rate. This suggests that preventing lung injury was more important than improving oxygenation. Many interventions have been shown to improve oxygenation in mechanically ventilated patients, but not to improve survival. Using lactate levels is an appealing method of determining whether oxygen delivery is adequate, but it has its limitations as well. Most lactate production in critical illness is not due to anaerobic metabolism, despite common assumptions. Instead, it is a product of increased pyruvate production (with metabolism to lactate) in the setting of impaired or altered glycolysis and gluconeogenesis. Lactate is the preferred fuel for cardiac myocytes in the setting of adrenergic stimulation and is produced by aerobic cellular respiration. Thus, lactate should be viewed as a nonspecific marker of physiologic stress. If the lactate comes down following intubation, fluid resuscitation, etc., then it simply indicates that the patient is responding to therapy. It doesn’t imply restoration of aerobic metabolism in previously anaerobic tissues. Likewise, an increasing lactate may indicate that the patient has a condition that is leading to an increase in sympathetic tone and cortisolmediated stress response. Increasing oxygen delivery may or may not help the situation—it depends on what the underlying condition is. This concept leads to the fifth rule of oxygen: SaO2, SvO2, O2ER, and lactate are all pieces of information and not goals in themselves. They must be taken into account along with urine output, peripheral perfusion, mentation, and other clinical information before any treatment decisions can be made. Oxygen Toxicity The idea that supplemental oxygen can be toxic, especially in high doses, is not new. In neonates, high FiO2 has been associated with retinopathy and bronchopulmonary dysplasia. In adults, there is evidence of worse outcomes with hyperoxia in the setting of acute myocardial infarction and following cardiac arrest. High FiO2 in adults can cause irritation of the tracheobronchial tree and absorption atelectasis (due to the oxygen being absorbed without the stabilizing effect of nitrogen gas, leading to alveolar collapse). This is discussed in more detail in the chapter on “Safety Limits and Lung Protection.” The bottom line is that avoiding hyperoxia is easy and can be accomplished by reducing the FiO2. Even normoxia may not be necessary, and it may be prudent to tolerate a degree of permissive hypoxemia in order to avoid exposing the patient to high FiO2 or ventilator pressures. Remember that cardiac output has a much more significant effect on oxygen delivery than the saturation and focus on signs of adequate or inadequate oxygen delivery rather than strictly following the SaO2 and PaO2. This approach leads us to the sixth and final rule of oxygen: give the patient just as much oxygen as he needs. This may be less than you think. Six Rules of Oxygen 1. The SaO2 is what matters, not the PaO2. 2. An increase in cardiac output can offset hypoxemia. 3. The SvO2 is low in low-flow states 4. The DO2:VO2 ratio, SvO2, and O2ER reflect the balance between delivery and consumption. They don’t represent a specific target for intervention. 5. SaO2, SvO2, O2ER, and lactate are all pieces of information and not goals in themselves. They must be taken into account along with urine output, peripheral perfusion, mentation, and other clinical information before any treatment decisions can be made. 6. Give the patient just as much oxygen as he needs. This may be less than you think. Chapter 6 Permissive Hypercapnia Permissive hypercapnia is the practice of allowing a mechanically ventilated patient to develop or remain in a respiratory acidosis rather than exposing him to the risk of injurious ventilator settings. For the purposes of this chapter, permissive hypercapnia is defined as a PaCO2 > 45 mm Hg with a pH < 7.35. Hickling et al. first described this concept in two papers that demonstrated a survival benefit with lower tidal volumes and elevated PaCO2 levels.3,4 This work was influential on later studies that showed the superiority of low tidal volume ventilation, including the landmark ARMA study performed by the ARDS Network investigators. Most of the studies examining this topic have focused on the benefit of using a lower tidal volume (4-6 mL/kg predicted body weight) in ARDS. There is less research on the benefits and risks of permissive hypercapnia itself, but there may be some advantages to permitting a mild to moderate respiratory acidosis in patients with severe respiratory failure. Pulmonary Benefits of Permissive Hypercapnia The primary rationale for hypercapnia is that avoiding iatrogenic ventilator-induced lung injury is more important that attaining normal gas exchange. Overdistension of healthy alveoli leads to cellular injury and is referred to as volutrauma. This is the primary mechanism of ventilatorinduced lung injury (VILI) and is independent of distending pressures (barotrauma). The ARMA study demonstrated a reduction in mortality in patients with ARDS when tidal volumes of 4-6 mL/kg PBW were used, compared with tidal volumes of 12 mL/kg.5 This benefit was seen despite worsening gas exchange in the low tidal volume group. In patients with status asthmaticus, using lower tidal volumes and respiratory rates prevents dynamic hyperinflation, pneumothorax, and pneumomediastinum, even though it may lead to a respiratory acidosis. Permissive hypercapnia is considered acceptable because the benefits of avoiding lung injury are considered far more important than achieving “normal” alveolar ventilation. Since current practice emphasizes the use of a low tidal volume in ARDS, increasing the tidal volume to correct a respiratory acidosis is seldom done. Instead, the respiratory rate is adjusted to increase or decrease the minute ventilation. Most of the time, increasing the respiratory rate on the ventilator is sufficient to blow off CO2 and normalize the pH. This may not be necessary, however, as patients are able to tolerate even a significant respiratory acidosis so long as oxygenation is maintained.6 In fact, there may be harm with this common practice. An increase in the frequency of tidal ventilation invariably leads to an increase in the cyclical opening and closure of vulnerable lung units. A patient with a set respiratory rate of 20 breaths per minute will have 11,520 more ventilatory cycles per day than another patient with a respiratory rate of 12 breaths per minute. Each one of those ventilatory cycles has the potential, albeit small, to contribute to VILI. Laboratory data supports the idea of using a lower ventilator rate whenever possible;7 however, prospective studies in humans will be needed to validate this concept. In the absence of data, though, it is certainly reasonable to question the necessity of routinely increasing the ventilator rate to correct mild to moderate acidemia. Extrapulmonary Benefits of Permissive Hypercapnia No prospective, randomized human trials examining the extrapulmonary benefits of permissive hypercapnia have been done. There are several laboratory studies in animals that have demonstrated a beneficial effect of hypercapnia on free radical production, myocardial injury, and cerebral ischemia.8 This reduction in pro-inflammatory cytokines and oxidative injury may prove to be helpful in reducing multisystem organ dysfunction, especially because the majority of patients with ARDS die of multisystem organ failure rather than of primary respiratory failure. In healthy human volunteers, controlled hypercapnia under general anesthesia was shown to increase both cardiac output and tissue oxygenation.9 A study of patients with severe ARDS demonstrated an increase in cardiac output and systemic oxygen delivery with a tidal volume reduction and hypercapnic acidosis;10 the same study, however, also showed worsening right ventricular function and hemodynamics. In a study of patients with subarachnoid hemorrhage and cerebral vasospasm, controlled hypercapnia led to an increase in cerebral blood flow without prohibitory elevations in intracranial pressure.11 While this is not sufficient to justify a change in recommended ventilator management, these findings do argue against the presumption of harm with respiratory acidosis during mechanical ventilation. Buffering In the ARMA study and subsequent ARDS Network studies, administration of buffering fluids was permitted to keep the pH ≥ 7.15. Sodium bicarbonate (NaHCO3) is often used to treat acidemia, but it does have several drawbacks. Under usual conditions, the bicarbonate anion is converted to CO2 and H2O via carbonic anhydrase: The elimination of the excess CO2 produced by this reaction is not normally an issue—one or two breaths are sufficient to clear it. In the setting of severe respiratory failure, however, elimination of the CO2 may not be possible, and the pH may in fact fall with the administration of sodium bicarbonate. In addition, CO2 diffuses freely over cell membranes (including in the CSF), but HCO3- does not. This has the effect of worsening intracellular acidosis, even if the systemic pH rises. A transient hemodynamic improvement is often seen when a bolus of sodium bicarbonate (e.g., an “amp”) is given, but this is more likely due to the loading of sodium than the change in pH—similar effects are seen with bolus dosing of hypertonic saline. Keep in mind that the NaHCO3 given in a 50 mL ampule is 8.4%, which is a very hypertonic sodium solution. THAM (tris-hydroxymethyl aminomethane) is a direct H+ ion buffer that does not depend on alveolar ventilation like NaHCO3. It also crosses cell membranes freely and produces intracellular buffering. This may be a more effective buffer for hypercapnic acidosis, but there are scant clinical data for its efficacy. At the time of this writing, the point is moot—THAM has been discontinued by the only manufacturer that was producing it. The necessity for buffering a respiratory acidosis during mechanical ventilation is debatable. The purported benefits of permissive hypercapnia (beyond the prevention of volutrauma) may be lost when the pH is increased. Administration of sodium bicarbonate may have some adverse effects, as described above, and there are no available non-bicarbonate buffers available for clinical use. Additionally, acidemia may confer a protective effect on hepatic and renal function, and systemic acidosis shifts the oxygenhemoglobin dissociation curve rightward, thereby augmenting tissue oxygen delivery. Using buffer therapy to keep the pH ≥ 7.15 is a common, but unproven, practice. Until clinical studies show a benefit to doing this, it would be prudent to reserve buffering for situations where the clinician feels that the acidosis is having an adverse effect on the patient. Downsides of Permissive Hypercapnia Despite the aforementioned benefits, there are some clinical downsides of hypercapnic acidosis in critically ill patients. The most widely recognized is the correlation between hypercapnia and intracranial hypertension. Hypercapnia does lead to vasodilatation, including cerebral vasodilatation. While this may augment cerebral oxygen delivery,11 it also increases the intracerebral blood volume. If intracranial compliance is diminished, this can lead to higher intracranial pressure. This may or may not be dangerous, depending on the degree of intracranial hypertension, but it certainly bears consideration. If hypercapnia is unavoidable in a patient with significant brain injury, then intracranial pressure monitoring should be considered. In patients with both acute and chronic pulmonary hypertension, hypercapnia can cause higher pulmonary artery pressures and lead to right ventricular dysfunction. Much of this is due to the underlying lung disease, but if there is clinical evidence of impaired hemodynamic function, then lowering the PaCO2 may be beneficial. Other systemic effects of hypercapnia are more related to the resulting acidosis than from the effect of the PaCO2 itself. These include impaired cardiac contractility, prolonged QT interval, decreased systemic vascular resistance, and hyperkalemia. If these occur, then buffer therapy may be warranted if other methods of correcting the respiratory acidosis would result in lung injury. On a microcellular level, hypercapnia has been associated with increased tissue nitration and the production of peroxynitrite. This radical is released during conditions of physiologic stress and may mediate tissue damage.12 The significance of this in clinical medicine is yet to be determined. Neutrophilic activity against bacterial infection is also attenuated with hypercapnia, but this can be overcome with the administration of antibiotics.13 Summary and Recommendations Permissive hypercapnia is a proven strategy for reducing VILI in patients with severe respiratory failure, be it due to ARDS or obstructive diseases like asthma or COPD. To state it simply, it’s more important to prevent iatrogenic lung injury than to get “normal” gas exchange. The degree to which permissive hypercapnia has a clinical benefit beyond preventing volutrauma remains to be seen, but the existing literature suggests that this may be the case. Chapter 7 Monitoring the Ventilated Patient Minute-by-minute monitoring of critically ill patients is a key part of the Intensive Care Unit. As discussed earlier, the respiratory status of a patient can be evaluated with clinical examination, a chest X-ray, and an arterial blood gas. These aren’t continually performed, however, and we need tools that allow us to know immediately when there is a change or deterioration in the patient’s condition. Pulse oximetry is now used universally to monitor oxygenation. Waveform capnography also can provide some key information and is very helpful, but it is not used on every ventilated patient. Pulse Oximetry Pulse oximetry uses two wavelengths of light—red, with a wavelength of 660 nm, and infrared, with a wavelength of 940 nm. Oxygenated hemoglobin preferentially absorbs the infrared light and allows the red light to pass through the tissues; deoxygenated hemoglobin, on the other hand, absorbs red light but not infrared light. When the sensor is placed on a highly vascularized but thin bed of tissue like a fingertip, earlobe, or forehead, the ratio of light absorption can be measured.14 This is converted to display a reading of the hemoglobin oxygen saturation (SpO2). If the sensor is applied properly and there is adequate perfusion, it is nearly as accurate as the SaO2 measured with an arterial blood gas analyzer. Pulse oximetry can be inaccurate if there is hypoperfusion from shock or significant vasoconstriction, either from shock or from vasoconstricting medications like phenylephrine or epinephrine. Generally, an inaccurate SpO2 reading due to hypoperfusion or vasoconstriction will also have a poor plethysmograph—in other words, the waveform will not be well-defined, and it will not correlate with the patient’s pulse. The SpO2 also becomes less accurate during significant hypoxemia (SaO2 < 80%), especially with darkly pigmented skin. Dyshemoglobinemias can also affect the accuracy of the SpO2. Carbon monoxide binds avidly to hemoglobin and absorbs infrared light readily, which leads to a falsely high SpO2 (98-100%, even with significant arterial hypoxemia). Methemoglobin, on the other hand, affects both infrared and red light absorption and can alter the SpO2 to underestimate the SaO2 with lower levels of methemoglobinemia. At higher levels of methemoglobinemia (> 35%), on the other hand, the SpO2 tends to read 80-85% no matter what the PaO2 or SaO2. Co-oximetric blood gas analysis is the best way to diagnose dyshemoglobinemias and to see what the true PaO2 and SaO2 are. Massimo has developed a noninvasive pulse oximeter that uses additional light wavelengths to accurately display the fraction of oxyhemoglobin, carboxyhemoglobin, and methemoglobin. There is one situation where the SpO2 is more accurate than the SaO2 (the ABG measurement). In hyperleukocytosis due to leukemia (WBC > 50K) or extreme thrombocytosis (platelet count > 1M), activated leukocytes or platelets will continue to consume oxygen in the blood gas syringe before it’s placed in the analyzer, leading to falsely low PaO2 and SaO2 measurements. Cooling the ABG sample on ice may reduce this, but in general the SpO2 is more accurate than the SaO2 when the WBC or platelet count is extremely high. Causes of Inaccurate Pulse Oximetry Measurements
Carbon Monoxide Poisoning: the SpO2 will read high (98100%) despite significant arterial hypoxemia. Methemoglobinemia: with significant methemoglobinemia, the SpO2 will read 80-85% no matter what the SaO2 or PaO2 are. Vasoconstriction: can lead to falsely low SpO2 readings;
the waveform is usually poor and not correlated with the patient’s pulse. Darkly Pigmented Skin: can lead to inaccurate SpO2 readings, but this is rarely seen except when the SpO2 is < 80%. Capnography Capnometry is the measurement of exhaled carbon dioxide in numerical form, usually measured in mm Hg. Capnography is the same information, but in graphical form. In addition, the capnograph will show the pattern of ventilation. Capnography is becoming a widely accepted practice in the ICU. Carbon dioxide is the major product of metabolism, and the exhaled CO2 tension can be used to make assessments of the body’s circulatory and ventilatory status. Most (60-70%) carbon dioxide is carried in the bloodstream in the form of bicarbonate ion— carbonic anhydrase in the RBC accounts for this. 20-30% is carried bound to proteins and hemoglobin. This leaves 5-10% to exist as dissolved carbon dioxide, which can be measured as the PaCO2. This is the usual measurement of ventilation. The capnograph can provide measurement of the end-tidal CO2 (ETCO2), which, when used with the PaCO2, can yield a great deal of information. Breaking Down the Capnograph A. Respiratory Baseline: This should be at zero. This part of the capnograph indicates the exhalation of CO2-free air—the air in the non-ventilated parts of the tracheobronchial tree. Elevation of the baseline indicates rebreathing of exhaled CO2. When the baseline and the ETCO2 rise, this usually means the sensor is contaminated. B. Expiratory Upstroke: This should be a steep slope, as it indicates the rapid exhalation of CO2 from the proximal acini mixed with deadspace gas. When it is less steep, this indicates a prolongation of the time it takes for CO2 to get from the acini to the sensor. This can be seen in cases of bronchospasm and incomplete obstruction of the endotracheal tube from secretions. C. Expiratory Plateau: This should be horizontal, or nearly so. The end point of the plateau is the ETCO2. This phase indicates the maximal ventilation of CO2 from the lungs. An up-sloping plateau can represent incomplete alveolar emptying, such as seen in COPD, partial airway obstruction, or obstruction of the endotracheal tube. D. Inspiratory Downstroke: The fourth phase represents the inhalation of CO2-free gas. Leaks in the ventilator system or cuff can cause prolongation of this phase. Clinical Use of Capnography The first step in interpreting the capnograph should be to assess the presence or absence of a waveform. The absence of a waveform indicates one of two things: 1. Failure to ventilate (esophageal intubation, dislodged endotracheal or tracheostomy tube, obstruction of the tube, apnea). THIS IS AN EMERGENCY. FIX THE PROBLEM. 2. Mechanical mishaps—fix the equipment! This should only be considered after you’ve thoroughly investigated #1, by the way. The CO2 sensor can get contaminated with mucus secretions, blood, or water and may become faulty. Equipment failure is a diagnosis of exclusion once more serious causes have been ruled out. Keep in mind that the ETCO2 will never exceed, and rarely match, the PaCO2. The ETCO2 isn’t a replacement for the arterial blood gas. Knowing that the ETCO2 never exceeds the PaCO2 is very helpful—it means that whatever the ETCO2, the PaCO2 is at least that high. It could be a little higher, or a lot higher, but it’s higher. For example, if a patient has an ETCO2 of 60, you know that the PaCO2 is at least that. It could be 65, or 105, but it’s at least 60. If it’s important to the patient to have a PaCO2 in the 35-40 range, then you know to increase the minute ventilation on the ventilator. The next step is to compare the ETCO2 with the PaCO2. The normal arterial-alveolar CO2 gradient is usually 3-5 mm Hg. A wide gradient means that there are areas of lung where the ventilation and perfusion aren’t matched. Consider the following if there is a large difference between the PaCO2 and ETCO2: Poor cardiac function, leading to low perfusion Pulmonary embolism, leading to an increase in dead space ARDS VQ mismatch from COPD, pneumonitis, or other pulmonary pathology Hypovolemia and/or hemorrhage Air trapping from dynamic hyperinflation Overdistension of alveoli from excessive ventilator pressure It’s just as important to follow trends in the gradient. A gradient of 10 mm Hg represents a significant change in a patient who had a PaCO2-ETCO2 gradient of 4 mm Hg yesterday. Look at the waveform and its amplitude—a discernable waveform with low amplitude usually represents a sudden increase in dead-space ventilation. However, even cardiac arrest will not drop the plateau to zero—the CO2 in the lung must be washed out. A zero or near-zero reading, especially with an abnormal waveform, usually indicates a misplaced ETT, obstruction of the tube, or an equipment problem. A waveform with a gradually decreasing amplitude indicates diminishing CO2 production (hypothermia) or decreasing CO2 delivery to the lungs (cardiac failure). A sudden increase in the ETCO2 occurs when there is an abrupt increase in CO2 production. This can occur with hyperthermia, after a seizure, bicarbonate administration, reperfusion of an ischemic limb, or ROSC after cardiac arrest. With severe bronchospasm and other causes of increased airway resistance, there is incomplete emptying of the alveoli during exhalation. This means that the capnograph will not level off as it normally should, and instead it looks more like a shark fin. If you see this, evaluate the patient for high airway resistance. Capnography During Cardiopulmonary Resuscitation CPR is often an unscientific, throw-the-book-at- ‘em procedure. Capnography is a cheap, noninvasive, objective method for assessing the success or failure of various interventions. Cardiac arrest causes a wide gradient between the arterial and exhaled CO2, due to lack of pulmonary blood flow. Narrowing of the gradient, as demonstrated by rising ETCO2, indicates increasing pulmonary circulation and therefore increasing cardiac output. Animal and human studies have shown the correlation between ETCO2 and coronary perfusion pressure, the chief predictor of ROSC (return of spontaneous circulation). It also has been shown to correlate with cerebral blood flow. Transient increases in the ETCO2 can be seen with administration of bicarbonate and high-dose epinephrine. Return of spontaneous circulation will produce a sudden increase in ETCO2, usually to 40 mm Hg or more. One major advantage of using capnography in CPR is the ability to assess the efficacy of chest compressions. A drop in the ETCO2 can be due to rescuer fatigue. ETCO2 can also be used to guide the depth and frequency of chest compressions—this is objective data, and much more reliable than pulse palpation (usually retrograde venous flow). If the ETCO2 is less than 10 mm Hg, the American Heart Association’s ACLS Guidelines suggest trying to improve CPR quality by optimizing chest compressions. Additionally, a discernable waveform and ETCO2 may reflect pseudo-electromechanical dissociation, which should be treated with vasopressors and fluid support. Interpretation of Changes in ETCO2 Rising ETCO2, Rising PaCO2: Alveolar hypoventilation due to oversedation, diminished respiratory drive, or neuromuscular weakness. Increased CO2 production from hyperthermia, thyrotoxicosis, reperfusion, ROSC following cardiac arrest, etc. Falling ETCO2, Falling PaCO2: Alveolar hyperventilation due to pain, agitation, fever, etc. Falling ETCO2, Unchanged or Rising PaCO2: Increased pulmonary dead space due to pulmonary embolism, worsening cardiac function, bleeding, hypovolemia, or dynamic hyperinflation with autoPEEP. Chapter 8 Safety Limits and Lung Protection Since the advent of mechanical ventilation, there has been concern that the technology could be harmful rather than helpful. Positive pressure-related lung injury was described by Madge and Charles Macklin in 1944, which was confirmed by inflating calf and cat lungs to the point where pulmonary interstitial emphysema and pneumomediastinum occurred. They also correlated these observations with patients who were tracheotomized and given assisted breathing during surgery and made the astute observation that a transpulmonary pressure of about 25 cm H2O was associated with lung injury. In the summary of the article, the Macklins note that “(w)omen in childbirth probably make much greater efforts than most men in their work,” which contrasted with their findings that pulmonary interstitial emphysema was more common in men (but remains a true statement!).15 In 1970, Mead described the concept of “stress risers,” where heterogenous alveoli could be exposed to different distending pressures within the same lung. This work introduced the concept of barotrauma with positive pressure mechanical ventilation, which was by then the standard treatment for acute respiratory failure.16 Four years later, Webb and Tierney demonstrated that rats, when ventilated at high airway pressures (45 cm H2O), rapidly became cyanotic and died of hemorrhagic pulmonary edema. They also found that application of end-expiratory pressure greatly ameliorated the damage, which they ascribed to surfactant depletion.17 In 1988, Dreyfuss published another rat study where they bound the rats with wire and then ventilated them with different volumes. This study supported the idea of using end-expiratory pressure to reduce lung edema; additionally, using a lower tidal volume was better than a high tidal volume in the animal model, irrespective of airway pressure. This research helped lay the foundation for our understanding of lung-protective ventilation and the mechanisms of ventilator-induced
키워드에 대한 정보 the ventilator book pdf
다음은 Bing에서 the ventilator book pdf 주제에 대한 검색 결과입니다. 필요한 경우 더 읽을 수 있습니다.
이 기사는 인터넷의 다양한 출처에서 편집되었습니다. 이 기사가 유용했기를 바랍니다. 이 기사가 유용하다고 생각되면 공유하십시오. 매우 감사합니다!
사람들이 주제에 대해 자주 검색하는 키워드 The best ICU book references for new providers
- AGACNP
- AGACNP student
- ICU references
- Best ICU books
- Critical Care Books
- Best ICU resources
- Ventilators
- Critical Care
- RN
- NP
- Nurse
- New ICU provider
- Resources for ICU newbie
- New grad NP
- Resident
- Medical Resident
- PA
The #best #ICU #book #references #for #new #providers
YouTube에서 the ventilator book pdf 주제의 다른 동영상 보기
주제에 대한 기사를 시청해 주셔서 감사합니다 The best ICU book references for new providers | the ventilator book pdf, 이 기사가 유용하다고 생각되면 공유하십시오, 매우 감사합니다.